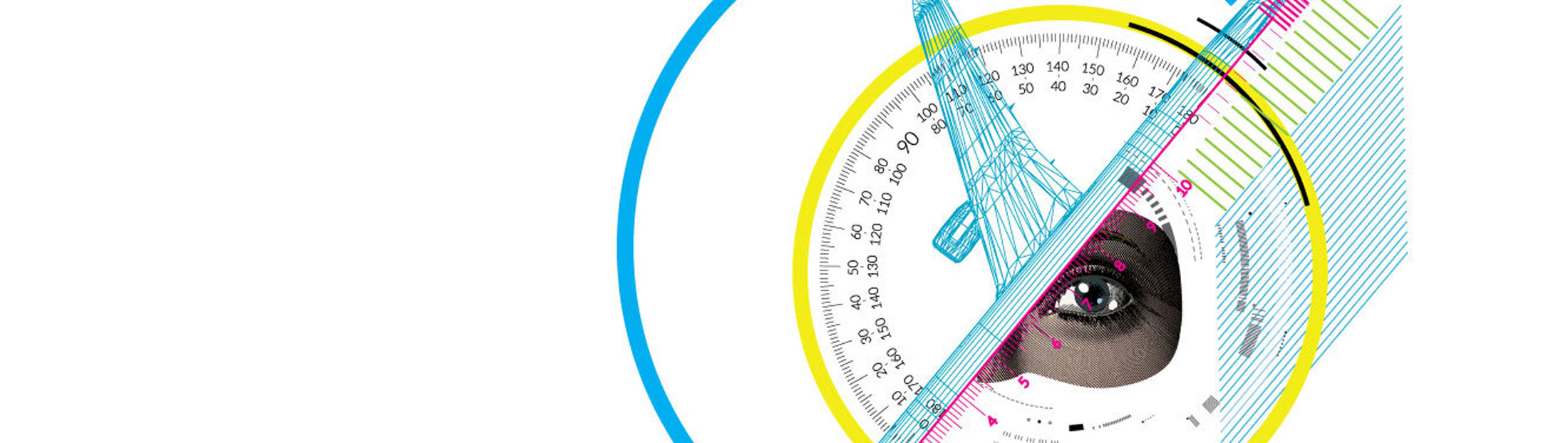
The Future of Lasers
Story by Colin Barras
Story by Colin Barras
You could be forgiven for thinking physicists are obsessed with monumental architecture. Although their investigations often involve objects at the subatomic scale, the machines they use can be enormous. In 2008, for instance, construction work finished on the Large Hadron Collider (LHC), straddling the Swiss-French border. Sometimes described as the world’s largest machine, the LHC is a 27-kilometer-long circular accelerator that cost several billions. Already there is talk of building even larger particle accelerators. Early in 2019, some of the physicists who helped build the LHC floated the idea of a new circular accelerator 100 kilometers long.
But there is now a new kind of scientific machine on the horizon: a trimmed down particle accelerator that is small enough to fit on a tabletop. Diminutive they may be, but these table-top accelerators are still bursting with the power and versatility scientists demand to perform cutting-edge research. Already they have helped physicists better understand the 3D-printed metal components that could one day be used in aircraft manufacturing, and experiment with a new and improved way to hunt for cancer tumors. What’s more, the table-top devices should be so cheap to build that many universities may ultimately possess one. This means accelerator technology that is currently beyond the reach of many researchers may soon be far more widely available, which has the potential to speed up the pace of scientific investigation.
Developing these new tiny accelerators is a global endeavor, but researchers at U-M have made a particularly significant contribution. Some of the key technological breakthroughs that are paving the way for the new machines involve advances in laser technology that were made by past and present researchers in the College of Engineering – including a 2018 Nobel laureate.
Over the last century, particle accelerators have become among the most important instruments in the scientific toolkit. It’s vast accelerators like the LHC, where subatomic particles are smashed together, that often grab the headlines. But in many accelerators, the idea is to manipulate fast-moving particles without colliding them – a process that can generate beams of intense X-ray radiation. Those X-ray beams have many uses. They have proved their worth in pharmaceutical drug discovery, in semiconductor research, and even in fossil analysis: X-ray beams can help identify the remains of ancient pigment molecules and reveal the original color of long-extinct animals.
“If we could afford it, there would be [X-ray-generating accelerators] in every university,” says Zulfikar Najmudin, a Professor of Physics at Imperial College London, UK. “But it’s just not possible because they are very expensive.”
Typically, an X-ray-generating accelerator costs hundreds of millions of dollars to build and, once complete, occupies an area the size of several football fields. Both factors make investing in an accelerator impractical for most universities.
An unfortunate consequence of this is that the accelerators are also beyond the reach of most scientists. There are just too few of the costly machines worldwide to meet the demands of modern research, meaning many scientists performing important work can wait years to obtain access.
Alexander Thomas gives an example. Thomas is a U-M associate professor of Nuclear Engineering and Radiological Sciences (NERS) in the Center for Ultrafast Optical Science (CUOS), as well as an associate professor of Electrical and Computer Engineering (ECE) and an associate professor of physics. He says additive manufacturing, also known as 3D printing, is becoming an increasingly important technology. “We’re to the point now that you can 3D print metals, and print components for aircraft,” he says. But tiny imperfections in the printing process could reduce the strength of those critical components. “You really don’t want these pieces to fail.”
One way to assess the quality of 3D printed parts is to probe them with the X-ray beams generated by an accelerator. But it’s difficult to secure access to those accelerators for this kind of fundamental research. What’s needed is a cheaper – and therefore more plentiful and available – form of accelerator. It’s this gap in the market that could be plugged by table-top accelerators. Using early versions of the table-top technology at CUOS and in the UK, Thomas is already collaborating with material scientists to investigate the internal structure of 3D-printed parts and identify imperfections that will help the researchers work out how to finesse the printing process.
Given how useful table-top accelerators promise to be, it’s perhaps worth asking why they are still in the development stage, while larger accelerators have been operational for decades. The reason is that the smaller versions of the technology operate in a completely different way than their larger counterparts.
A traditional particle accelerator uses powerful magnets to gradually ramp up the speed of subatomic particles – typically negatively charged electrons – as they travel through a ring-shaped vacuum chamber that is often hundreds of meters long. The miniature version effectively replaces this large, circular race track with a drag strip measuring just a few tens of centimeters in length. Over that short distance, electrons are accelerated at a far higher rate. That acceleration process is triggered by astonishingly brief and intense laser pulses. This means it has only become possible to think about table-top accelerators because of advances in laser technology.
The first working laser was demonstrated in 1960. Within a few years, physicists had discovered that their lasers could be used to generate exceptionally short pulses of light, each lasting just a few trillionths of a second (or a few picoseconds). “At the time there maybe wasn’t a technological reason for making those short pulses,” says Karl Krushelnick, director of CUOS and, like Thomas, a professor of NERS, ECE and physics. “There was just an interest in seeing what you could do with lasers.”
As the years passed, physicists realized those short laser pulses could prove useful, but only if the researchers could find ways to increase their brightness (or intensity). Light has both electrical and magnetic properties, and so if a very brief flash of laser light is bright enough, it can generate a powerful electric field that will interact with any electrons in its path.
Unfortunately, generating short laser pulses of the required brightness had the potential to damage the laser equipment. In the mid-1980s, Gérard Mourou and Donna Strickland, working at the University of Rochester, developed a solution to this problem. Their method, Chirped Pulse Amplification, involved taking the short laser pulses and stretching them out in time, effectively diluting them so their brightness could be increased without damaging the hardware. Once boosted, the laser pulses were compressed again to give a very short – and now also very bright – laser pulse.
Within a few years, the researchers had refined the technology so it was possible to generate ultrashort pulses with intensities measuring 1018 watts per square centimeter. That’s the equivalent of many thousand times the output of all the world’s electricity power plants squeezed into an area one-quarter the size of a typical postage stamp. The research was so important it earned Mourou and Strickland a share in the 2018 Nobel Prize in Physics.
“We proved that we could increase laser intensity by orders of magnitude,” Strickland explained in a post on the Conversation, describing how she built the laser as Mourou’s graduate student. “In fact, CPA led to the most intense laser pulses ever recorded.”
Mourou moved to U-M in 1988 – a decision he made because of the university’s strong reputation. “U-M was very big in optics even in the 1960s,” he says. Mourou then helped secure a prestigious National Science Foundation grant to establish CUOS. During Mourou’s time at Michigan, U-M’s international reputation for ultrafast optics research grew. Several commercial companies had their roots in CUOS, including Intralase – a firm that used laser pulse technology to develop a virtual scalpel for vision-correction eye surgery.
But arguably, the culmination of Mourou’s work at U-M was the construction of the HERCULES laser system in the late 90s and early 2000s. Harnessing Chirped Pulse Amplification, HERCULES was, by 2004, producing 45 terawatt pulses of laser light with a world-record intensity of 1022 watts per square centimeter. It is lasers such as U-M’s HERCULES – and a similar UK laser facility called Astra Gemini – that power the table-top accelerator experiments now being conducted by Thomas, Krushelnick and Najmudin.
Before table-top accelerators became a genuine possibility, though, there was one more hurdle to overcome. By the early 2000s, physicists could use lasers like HERCULES to accelerate electrons to high speeds over very short distances. But those speedy electrons tended to shoot off in all directions. Physicists needed to tame them – corral them into narrow beams in which all of the electrons travelled along the same path at the same energy.
By 2002, researchers including Krushelnick, Najmudin and Stuart Mangles, a Reader in plasma physics at Imperial College London, had worked out how to encourage the electrons to form tight beams, but the electrons within those beams still carried different energies. “They were ugly beams,” says Thomas.
Then, in 2004, Mangles, Krushelnick, Thomas and Najmudin helped overcome this stumbling block. They were members of one of three research teams that independently worked out how to guarantee the electrons in the beams all carried the same energy. The research, which was conducted using the Astra Gemini laser in the UK, was such a significant step in the development of table-top accelerators that it made the cover of the prestigious Nature scientific journal.
“Those studies were truly enabling,” says Gianluca Sarri, a Reader in the School of Mathematics and Physics at Queen’s University Belfast, UK, who now collaborates with Krushelnick, Thomas and Najmudin.
It’s research that has taken them on a virtual voyage into the core of violent explosions occurring in galaxies far, far away.
Back in 2004, Krushelnick and Thomas were based at Imperial College London, but a few years later they both moved to U-M. The HERCULES laser was a major factor in that decision, says Thomas. “It’s not just that it has such a high intensity,” he says. “It’s having a laser of that scale in a university setting – that’s quite unique.”
Over the last decade, the researchers have begun putting their laser-powered table-top accelerators to work, addressing fundamental questions at the cutting edge of science. It’s research that has taken them on a virtual voyage into the core of violent explosions occurring in galaxies far, far away.
During the Cold War, suspicions were high that parties who had signed up to the 1963 Partial Nuclear Test Ban Treaty might attempt to break the agreement and conduct clandestine nuclear weapons tests in space, perhaps on the far side of the moon where they would be out of sight of Earth-based observers. United States authorities launched satellites in the 1960s and 70s to monitor for the gamma rays that the explosions would generate. In the late 1960s, the satellites began detecting such signals. They were not coming from behind the moon, however: they originated from deep space.
The earliest media reports speculated that these “gamma ray bursts” might be evidence of battles being fought between powerful alien civilizations in distant galaxies. As recently as the 1990s, some astronomers were suggesting that gamma ray bursts might be some sort of alien communication system.
“It’s actually not something to be taken too lightly,” says Sarri. “We do constantly receive a lot of signals from outer space. You have to try to catalogue them all and find a natural reason for them, so you can rule out that they are not alien transmissions.”
The consensus view today is that gamma ray bursts are nothing to do with extraterrestrials. But to really confirm the idea, astrophysicists need to know that their theoretical models for how the bursts form match with reality. This is where the table-top accelerators in Michigan and the UK enter the story. Using them, a team including Sarri, Krushelnick, Mangles, Najmudin and Thomas essentially generated mini-gamma ray bursts in the laboratory. Their research strongly suggests that at least some of the gamma-ray bursts that have been puzzling scientists since the 1960s stem ultimately from the behavior of black holes. “We now have other experiments lined up to probe the physics in more detail,” says Sarri.
The gamma ray burst experiments are an example of how the high-speed electrons from a table-top accelerator can be put to work. But in conventional accelerators it’s the X-rays these electron beams can produce that are arguably even more useful. It turns out that table-top accelerators can generate X-ray beams too, and they could transform healthcare.
When electrons are moving at high speed, any sudden changing in their direction of travel generates a bright beam of X-rays. Often, physicists use a special piece of hardware – a device they call a “wiggler” – to induce this direction change. With a table-top accelerator, however, the wiggler comes already included. Najmudin, Krushelnick, Thomas, Mangles and their colleagues discovered that when their laser pulses accelerate electrons, the particles are violently shaken at the same time. In other words, the electrons suddenly changes their direction of travel, which means they fire out an X-ray beam. “And the X-ray beam is actually really high quality,” says Najmudin.
It’s these X-ray beams that Thomas is now using to probe the internal structure of 3D printed materials that might eventually be used in aircraft manufacture. But the X-ray beams have other applications too. “We’re very interested in imaging tumors,” says Najmudin.
Already, doctors use low-energy X-ray beams to produce mammograms and search for breast cancers. But mammogram images are relatively fuzzy and poor quality. “It’s a real skill to be able to identify tumors, and there is a problem of false diagnosis,” says Najmudin. The higher quality X-rays generated by laser systems can generate sharper, better images.
This is because, using those higher quality X-rays, it’s possible to focus on phase contrast – the subtle but detectable way that X-rays change direction as they move through body tissues of slightly different compositions. In 2018, Najmudin and Mangles and their colleagues showed how this “phase-contrast imaging” could produce exquisite images of the different tissue types in a 1-centimeter-long mouse embryo. The same technique could one day make it easier to detect breast cancer tumors when they are still just a few millimeters wide rather than having to wait for them to grow to centimeter-widths. “That is probably one of the most exciting things we can now apply our research to,” says Najmudin.
Already, table-top accelerators are beginning to transform science. By boosting the power and intensity of the HERCULES-style lasers that drive the machines, even more will be possible. For instance, Najmudin points out that more energetic laser systems will be able to produce X-ray beams with enough energy to scan for tumors inside living people instead of just within tiny tissue samples.
As such, many eyes are now focused on the Extreme Light Infrastructure (ELI), a European project initiated by Mourou in 2006. Earlier this year, the ELI-NP laser facility in Romania began producing 10-petawatt (10 million billion watt) laser pulses that will ultimately be capable of intensities of 1023 watts per square centimeter – some ten times more intense even than HERCULES can presently muster.
Mourou – now a Professor and Member of the High College at Ecole Polytechnique in Paris, France – expects ELI-NP’s laser pulses will prove to be a powerful new tool for exploring the subatomic world. He also anticipates exciting future laser applications beyond the table-top. For instance, laser pulses could help change the trajectory of pieces of space junk in Earth’s orbit, sending them into the atmosphere to burn up. Mourou also thinks that laser pulses could help “transmute” nuclear waste – literally changing the chemical elements it contains into different elements so that instead of remaining dangerously radioactive for thousands of years the waste becomes safe in a matter of minutes.
Exciting though these European-led projects and proposals are, they also highlight that North America has lost ground at the cutting edge of laser research. “At one point all the highest intensity lasers were based in the U.S., but the past 10 or 15 years have seen a shift to Europe and to Asia,” says Krushelnick.
But there are promising signs that U.S. authorities want to reverse that trend. A 2018 report by the National Academies of Sciences, Engineering and Medicine recommended the Department of Energy consider building new laser facilities to rival those now being constructed in Europe and Asia. “Hopefully we might even leapfrog those facilities,” says Krushelnick.
There are reasons for near-term optimism too. In September 2019, the university announced an upgrade: with $16 million from the National Science Foundation, the work will soon begin on a major overhaul of the HERCULES laser system that will see it capable of producing 3-petawatt laser pulses. “That’s a factor of 30 increase in laser power and intensity over what we’ve had before, which puts us in the regime where we are able to produce antimatter from the laser beam interaction,” says Thomas. (See “Boiling the vacuum.”)
In this new upgraded form, HERCULES will be renamed ZEUS, and it should have a big impact on the continued development of table-top accelerator research at U-M. Mourou – who was instrumental in the initial construction of HERCULES – welcomes the developments. “I still keep in touch with [the CUOS researchers] and I’m extremely interested in seeing their progress,” he says.
As significantly, 2018 saw the establishment of LaserNetUS, a network that will help optics researchers across the U.S. form stronger collaborations and improve access to the HERCULES laser and the table-top particle accelerator experiments it allows. “It supports the running of these lasers to get the most out of them, and it’s also about building a community,” says Thomas. With LaserNetUS, and the NSF investment in the HERCULES/ZEUS laser, there is now a bright future for laser technology – and table-top accelerator research – across the U.S.