
It’s all on the table
Can engineers piece together a carbon-neutral aviation system by 2050?
Contents:
The fuel consumption per person for a Boeing 737 flying from Detroit to San Diego is the same as two people driving the same distance in a Prius,” Joaquim Martins, the Pauline M. Sherman Collegiate Professor of Aerospace Engineering, told the attendees of U-M’s Sustainable Aviation Symposium in September 2023, a statistic he’s quoted for more than a decade.
Then, he acknowledged that electric vehicles are on the verge of changing that calculation—a journey in an EV generates about 25% less carbon than hybrids emit directly, based on the average carbon emissions of the U.S. power grid. As the grid continues to transition to emissions-free power sources and charging technology brings fill-up time closer to that of gasoline-powered vehicles, environmentally-minded travelers may tolerate longer journeys for the moral advantage of low emissions travel.
This is the challenge that brought representatives from aircraft and engine manufacturing, airport and airline operations, NASA, the Federal Aviation Administration (FAA) and clean aviation startups to U-M to discuss the future of the field.
“For now, aviation produces only approximately 2% of global emissions. We might ask why not go after concrete, ground transportation, electrical generation,” said Carlos Cesnik, the Richard A. Auhll Department Chair of Aerospace Engineering and inaugural leader of the Michigan Initiative for Sustainable Aviation (MISA).
“But we are going after those, and as emissions from those sectors shrink, the share belonging to aviation will grow—especially if passenger numbers keep rising.”
Already, aviation accounts for 8% of U.S. greenhouse gas emissions associated with transportation, which is itself 29% of total U.S. emissions. Light duty vehicles like cars represent 58% of transportation emissions. If they converted entirely to EVs, even without gains in other sectors, aviation would account for nearly 20% of transportation emissions.
If we are not working on the solution today, there won’t be time tomorrow to start thinking about it.
Gökçin Çınar, assistant professor of aerospace engineering
Then consider that these numbers are from 2021—a low point for commercial aviation, not quite 60% of the global all-time-high in 2019. It’s easy to imagine that by 2050, aviation could be among the largest emitters in transportation. 2024 is expected to bring a record high number of passengers, and the UN’s International Civil Aviation Organization predicts average annual rises in demand of 4.3% into the 2040s.
In addition to that pressure, Jennifer Haverkamp, the Graham Family Director of U-M’s Graham Sustainability Institute, highlights the pressure from air passengers.
“The flying public has increasingly gotten the message that flying in planes today is a significant contribution to an individual’s carbon footprint,” she said, noting new words coined by the Swedes—flygskam (flight shame), tågskryt (train bragging), and smygflyga (flying in secret). They may be a European phenomenon for now, but airlines and airplane manufacturers are taking note.
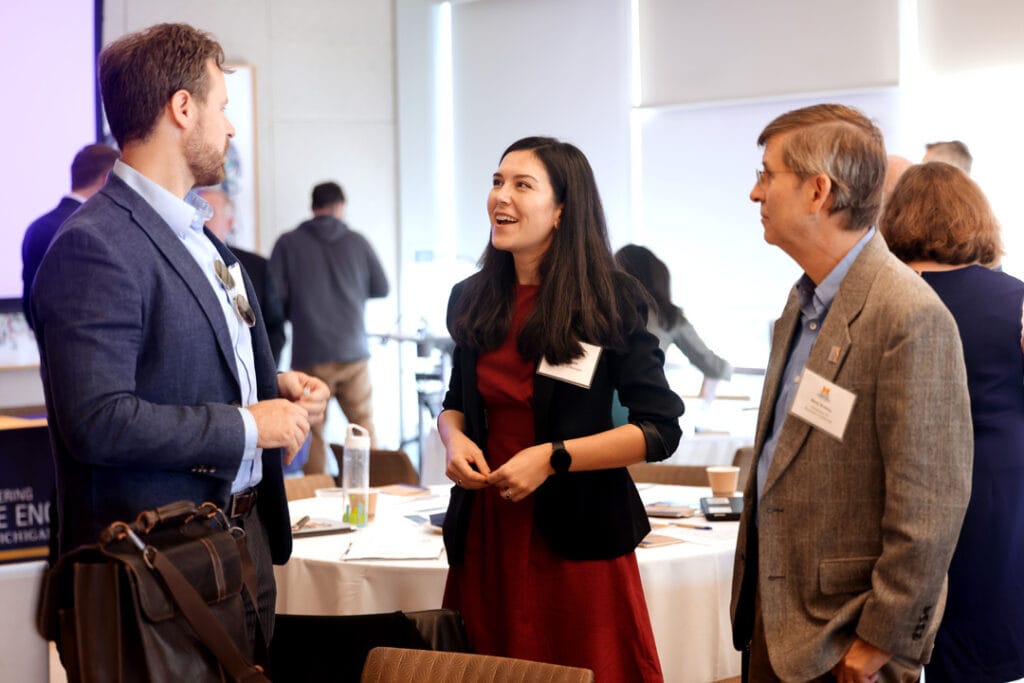
Moreover, the target date of 2050 for net zero emissions, needed to meet the 1.5 degrees Celsius warming target set by the Paris Agreement, feels uncomfortably close to aerospace engineers. Change in the aerospace industry comes extremely slowly; even iterative design changes like the Boeing 787 Dreamliner take nearly a decade to enter service. In addition, nearly every commercial aircraft uses the same basic technology that has been in service since the 1960s: a tube-and-wing design powered by jet engines or propellers running on kerosene-based fuel.
The slow pace is for good reason—the failure of a single component can put hundreds of people in danger, and hewing to similar designs means fewer risks of unexpected problems. Still, any truly transformative design, with major changes to the shape or propulsion mechanism of the aircraft, won’t be flying commercial passengers until the 2040s at the earliest.
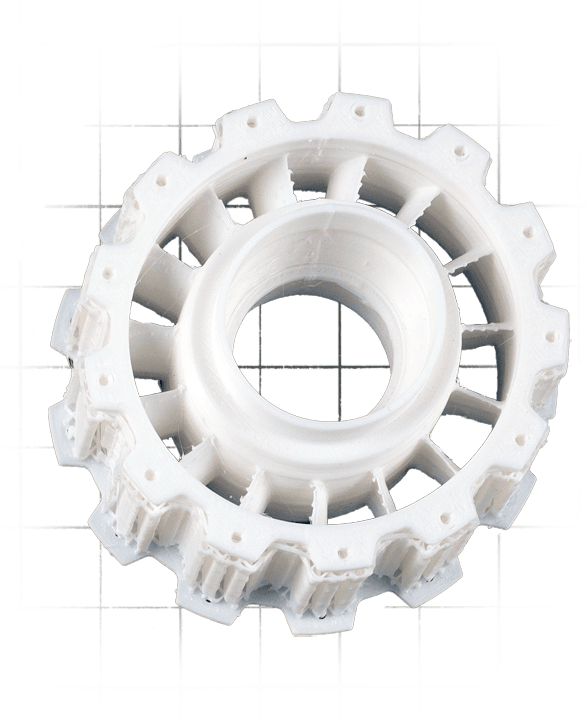
“2050 might seem so far away for other industries, but it takes about 10 to 15 years to design, build and mass manufacture an aircraft with conventional technology. Maturing and flight-proving novel technologies for decarbonizing will take much longer than that,” said Gökçin Çınar, a U-M assistant professor of aerospace engineering, who took the reins of MISA in early 2023. “We have just one shot. Maybe two, but that would be really cutting it close. So if we are not working on the solution today, there won’t be time tomorrow to start thinking about it.”
On the flip side, she says today is perhaps the most exciting time since the start of aviation to be an aerospace engineer. Rather than exclusively exploring incremental changes, commercial aviation is looking to make a calculated leap. That’s why Michigan Aerospace engineers started MISA: they see an opportunity to connect industry, airport authorities, government and regulatory agencies and academic research to help choose the right transitions and accelerate their adoption.
Aviation has nearly perfected kerosene-burning turbine engines and the tube and wing design—within the limits of what will fit at airport gates. It is generally accepted in aerospace engineering that the efficiency of commercial aircraft has improved by about 70% since the 1960s. This includes increases in engine efficiency as well as lighter and more aerodynamic airframes—and, of course, packing passengers in ever more tightly.
It’s darn near impossible to beat the efficiency of direct mechanical drive.
Matt Hutchison, Vice President and Chief Engineer, Boeing Vertical Lift Division
Cesnik talks about progress in reducing aviation’s emissions in terms of S-curves, the shape of technology evolution over time. Performance gains happen slowly at first as a technology becomes better understood, then quickly as it receives investment, and then slowly again as the low hanging fruit has all been harvested and gains are harder to make.
“We have gone about as far on the S-curve as we can. What we need to do is jump from one S-curve to the next,” Cesnik said.
The thing is, it’s hard to say what the next S-curve will be. Three technologies are currently vying for a place in the future of aviation: sustainable aviation fuels, electrification and hydrogen power—and there may be more than one winner. Even once the best options become apparent, building up the energy supply chain and replacing aircraft—with 30-year service lifespans—will require investment over decades.
“We are drawing on the benefits of roughly a century of slow infrastructure development since the first commercial jets took off, and we’re not going to scrap it overnight and build something else from scratch,” said Mirko Gamba, an associate professor of aerospace engineering and a member of MISA who is exploring combustion and propulsion.
Electrification and hydrogen have the potential advantage of emitting zero carbon, but they both have big hurdles to overcome. Batteries don’t have the energy density to fly large amounts of cargo or passengers. Hydrogen requires building a whole new infrastructure, from supply to distribution to new kinds of engines or even fuel cells. That makes sustainable aviation fuels (SAFs) the clear near term choice, since they require the least in new infrastructure and aircraft modifications. But it’s unclear how much of aviation’s energy needs they can ultimately fill.

The Eco Caravan, with its plug-in hybrid-electric powertrain, is now flying and available for pre-order. The 9-seater aircraft is powered by both a combustion engine and an electric propulsion system with energy supplied by a battery pack located in the cargo pod. Photo: Ampaire
Sustainable aviation fuels
“It’s darn near impossible to beat the efficiency of direct mechanical drive,” said Matt Hutchison, vice president and chief engineer of Boeing’s vertical lift division, at the Sustainable Aviation Symposium. “We are trying to replace modern turbines, which are incredible machines.”
Fifty percent efficiency. Extremely reliable. And they run primarily on kerosene, which has a high specific energy—the energy per unit mass. With kerosene at 6000 watt hours per kilogram, it’s easy to see why many aerospace engineers are skeptical that batteries, currently capping out at around 300 watt hours per kilogram, could ever compete. Even with an electric motor that is about 80% efficient, we’re still looking at an energy source that weighs more than 10 times as much and doesn’t get any lighter as the plane flies.
SAFs let us keep that high energy density while using most of the infrastructure we already have, with the possibility of a full shift to net carbon neutrality. SAFs can be made in many ways, including from food and yard waste, used oil and grease, forestry waste and ethanol.
ASTM International, a standards organization, already permits commercial flights to run on blends of up to 50% SAF mixed with conventional jet fuel. In 2021, United Airlines demonstrated the first passenger flight with 100% SAF in one engine, and in November of 2023, Virgin Airlines demonstrated the first transatlantic flight on 100% SAF.
However, we’re a very long way from an industry that can provide half of the 620 million barrels of jet fuel that U.S. aviation used in 2019, the most recent pre-pandemic year. In 2022, SAF production worldwide added up to less than 2 million barrels.
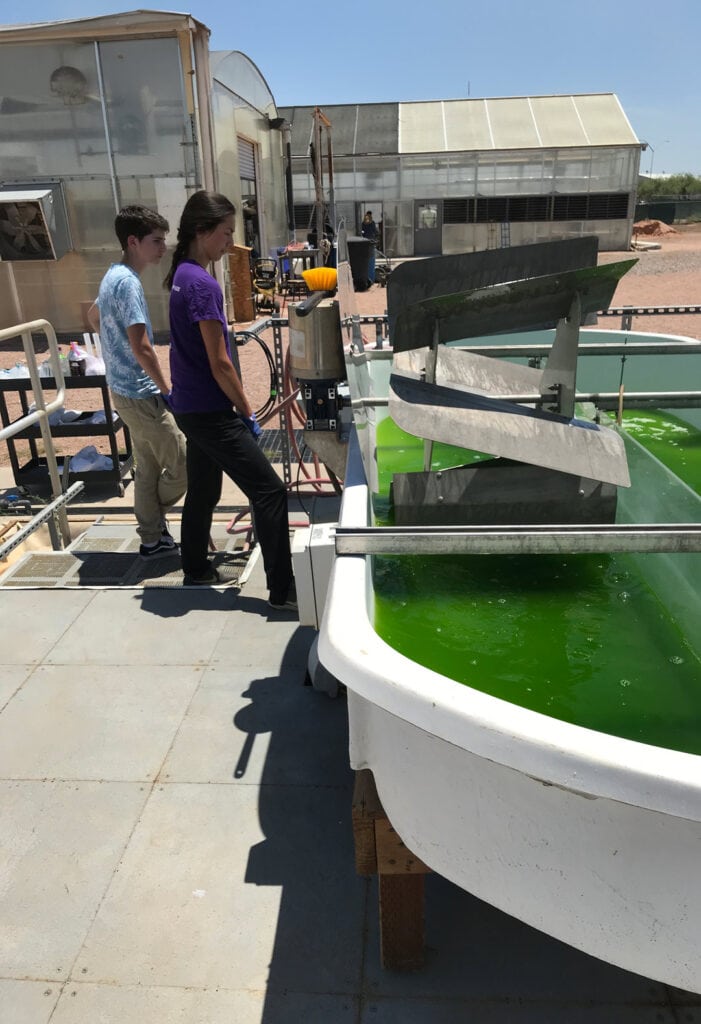
Gamba anticipates that the ramp-up of SAF will look a lot like that of ethanol for ground transportation, blending with conventional aviation fuel. This slow introduction will reveal issues that aren’t currently foreseen, similar to the incompatibility between ethanol and the hoses and other flexible components in machines designed for gasoline alone. As with ethanol, these components will be replaced with those that are compatible with SAF.
One might hope that as automobiles electrify, biofuel production could be freed up for aviation, but we already know cropland is not going to get us to 100% SAF. Today’s plant-based jet fuel is made mostly from soy, and U.S. aviation is projected to require 830 million barrels of jet fuel per year by 2050. To make that much soy-based biofuel, we’d need a land area spanning nearly a million square miles—the size of Alaska, California and Texas combined.
Biofuels made from algae, on the other hand, could produce the same amount of fuel in an area more than 90% smaller—closer to the size of New Hampshire. However, land-efficient algae biofuels have yet to be integrated into real-world fuel production.
Vennema Professor of Engineering André Boehman recently completed a $2 million project to grow algae, produce fuel and test how it burned. The research was featured in The Michigan Engineer in 2018. And while the fuel was of excellent quality, growing its algae feedstock proved to be a major challenge.
Interrupted by the pandemic-induced closure of the algae facility and Arizona monsoon rains that contaminated their pools, the team produced 300 milliliters rather than the two liters they’d intended. Still, the pre-pandemic batch was a success, and Boehman is pressing ahead with a new proposal for the production of SAF from algae that includes Gamba on fuel testing.
On the other hand, humanity may be moving toward the point where plants are no longer the most efficient and eco-friendly source of carbon for fuel. The Global CO2 Initiative, led by U-M, identified sustainable aviation fuels as an industry with a ready market for captured carbon dioxide. That carbon dioxide would be broken up and combined with hydrogen to produce liquid hydrocarbon fuels that could replace conventional jet fuel.
Carbon capture is often discussed in the context of smokestacks, air or water, but Volker Sick, the DTE Energy Professor of Advanced Energy Research at U-M and leader of the Global CO2 Initiative, pointed out that ethanol production may offer another source of carbon. The biofuel is criticized for releasing one third of the carbon captured in corn back into the atmosphere during fermentation. But ethanol plants are already installing carbon capture technology, and some of that carbon could one day fuel aviation.
“That CO2 is very pure, and it can be captured and converted to sustainable aviation fuel,” said Sick. “Thereby, you can really use most of the CO2 that plants have captured from air and put it into SAF in a very integrated way.”
The other raw material needed to make synthetic fuels is hydrogen, typically produced by splitting water. Likewise, Sick is keeping an eye on developments such as the hydrogen hubs launched by the U.S. Department of Energy.
Still, this possibility would need to rely on cheap, emissions-free electricity. And while using electricity to make synthetic fuels offers the same energy density as today’s fossil fuels, it’s much less efficient than simply using the electricity to power an electric motor.
“To make fuel from CO2 requires about four times as much energy as if you could use the electrons directly. But for long-haul aviation, direct use is not in the cards,” Sick said.
UPPING THE
AERODYNAMICS
Without a different energy source, aviation can’t reduce its carbon footprint enough to keep it from being one of the largest transportation emitters by 2050.
However, significant changes to the body of the aircraft could reduce fuel burn—reducing the battery weight needed, or the land needed to grow SAF, for instance.
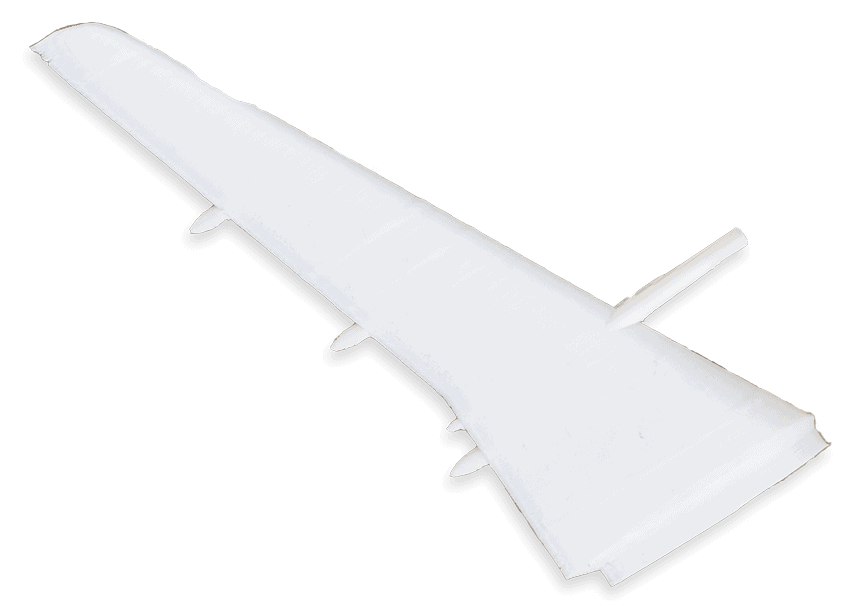
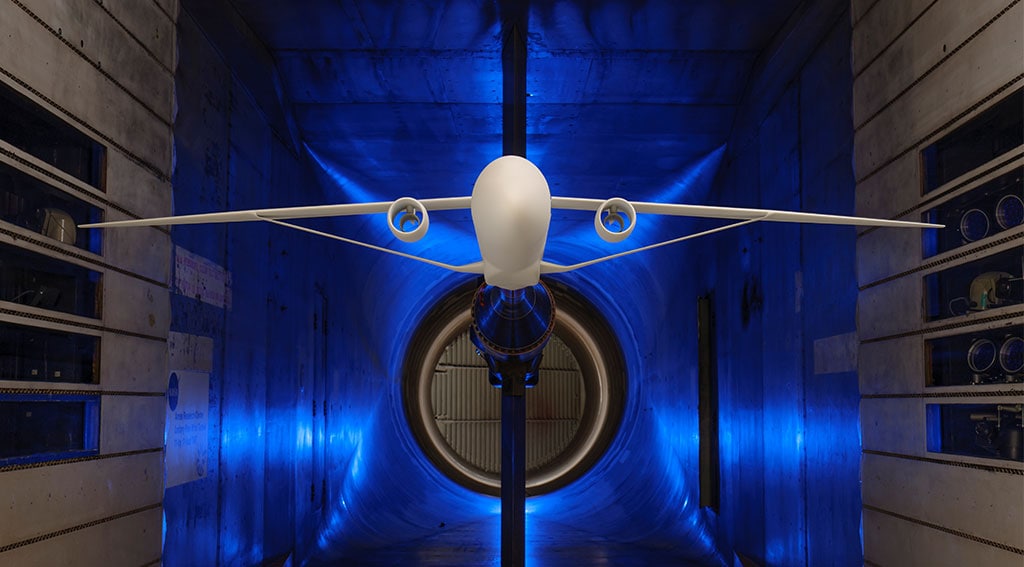
Longer Wings
FUEL SAVINGS
up to
10%
Longer wings can reduce fuel burn, particularly on long-haul flights when their better aerodynamics really pay off. But they come with a variety of challenges. One issue is that the longer wings don’t fit existing airport gates. Boeing introduced folding wingtips on its 777x as a
workaround, and wider gates could be a longer-term solution. A second issue with longer wings is their increased flex—a topic Airbus explored by funding a 5-year research center led by Cesnik. Boeing and NASA are trying out trusses to brace underneath the wings, reducing their flexibility.
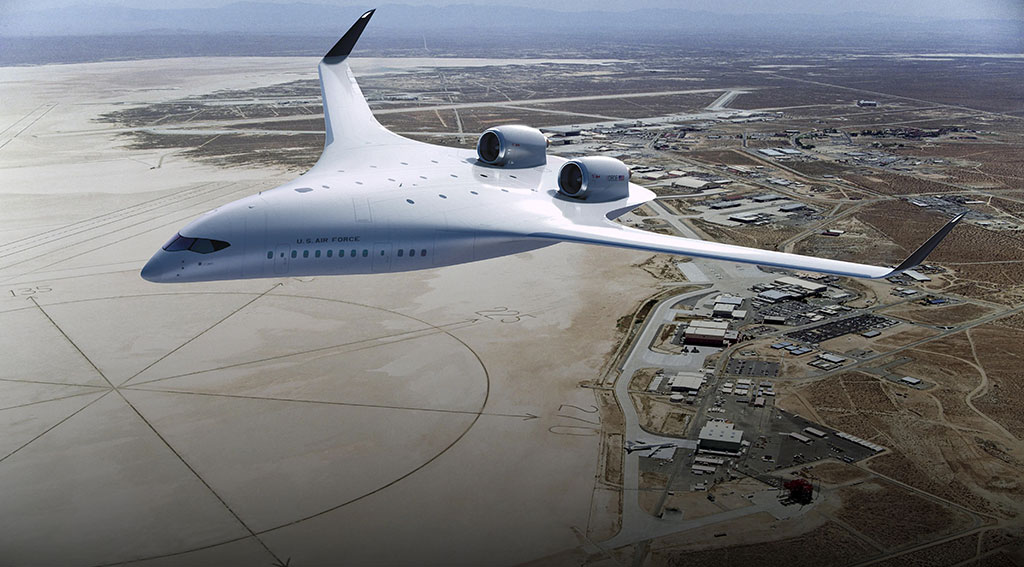
BLENDED-WING-BODY
FUEL SAVINGS
up to
20%
Also known as a flying wing, this design uses the entire surface of the plane to produce lift. This aerodynamic design has a shorter wingspan and also is quieter on takeoff and landing. However, it’s a major departure from the planes that pilots and airport mechanics are used to.
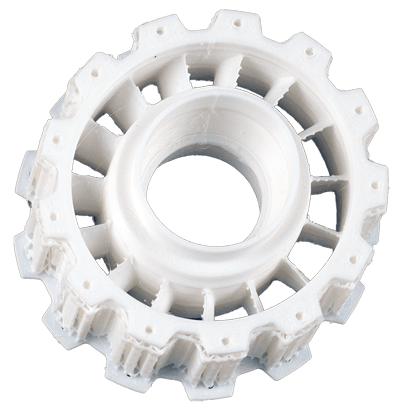
Electrification
Long haul flights relying on electricity alone are particularly difficult for aviation because batteries are so heavy—right now, they’d take up all the cargo and passenger space. But that’s not the only way to electrify. Aerospace engineers are experimenting with both hybrid and fully electric designs.
A hybrid setup would look different from the continuous storing and use of electricity that hybrid cars use. The aircraft power use pattern is enormous on takeoff and climb, smaller and steady during cruise and then minimal on the approach to land. Engines can produce more power than is needed for most of the flight, and their design is tilted even further from high-efficiency cruise to minimize the risk of crashing.
“For safety reasons, we require that when you size the engines that go in an airplane, you assume you’re going to lose one of them at takeoff. This way, you can still continue your climbing, go around and then come and land safely,” Cesnik said.
“So our engines become really oversized for the entire flight. If everything goes smoothly, we never use that amount of power available.”
He explained that hybrid planes might offer a way to fly safely without the need for fuel-guzzling oversized engines. Aerospace engineers are exploring propulsion solutions augmented with electric motors. The batteries would carry just enough energy to get through takeoff and climb, providing redundant power to enable a smaller main engine to get the plane to safety in the event of a failure. The smaller engines would burn less fuel during the cruise phase of the flight.
Çınar even suggested that it could be possible to run a turbine as a generator, without the weight of a battery, and do distributed propulsion with an array of electric motors. Her area of expertise is gaming out how different technologies perform in realistic situations: What designs optimize their performance at the system level, how they behave with other parts of an airplane, what kind of infrastructure support is needed, what fuel consumption and overall cost is expected, and so on. Studies like hers can try out different options virtually, anticipating challenges and weighing what technologies should move forward for which kinds of flights during the aircraft design process.
But even before those optimization simulations, engineers are anticipating challenges with running electric motors at high altitude. At the U-M Sustainable Aviation Symposium, Gaudy Bezos-O’Connor, project manager for the Electrified Powertrain Flight Demonstration project at NASA’s Langley Research Center, noted that the thin air of high altitudes isn’t as electrically insulating as the air on the ground. The high voltage circuits needed to deliver power to electrical motors would need extra insulation to prevent arcing. And of course, there’s the fire risk from the batteries themselves.
Then there’s the heat generated by the motors—20% of the energy that powers them.
“Electric motors generate a lot of thermal energy. Do we dump it or capture it in a regenerative cycle and use it for something else onboard?” asked Rickey Shyne, director of research and development at NASA’s Glenn Research Center, speaking at the symposium.
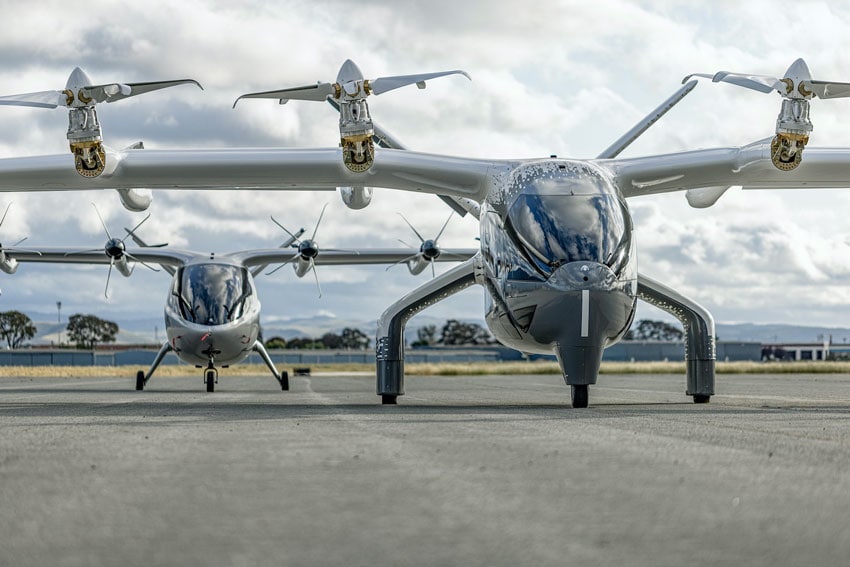
Already, batteries are enough for short hops to and from city centers, like the 12 miles from Chicago O’Hare International Airport to Chicago’s vertiport. United Airlines and Archer Aviation announced plans to begin this route with Archer’s electric air taxis in 2025.
Right now, Archer’s planes rely on batteries with nearly the same specific energy as a Tesla Model S-LR, roughly 150 watt-hours per kilogram. Battery researchers are looking to outdo that specific energy by more than six times, achieving a goal known as Batt1K—a battery that provides a thousand watt-hours per kilogram of mass. Because motors are more efficient than jet engines, Batt1K would provide about 25% as much thrust per kilogram of weight as jet fuel.
“It would enable a Boeing 737 that can go about 600 to 800 nautical miles. That’s the North Star goal that we’re shooting for,” said Venkat Viswanathan, an associate professor of aerospace engineering whose company, Propel Aero, was recently awarded $1 million by the Department of Energy to pursue a Batt1K concept.
Batt1K would enable larger commercial flights from Detroit to Washington, D.C., for example, or perhaps Chicago to Houston at a stretch. That could electrify roughly a third of commercial flights with a capacity of 150 passengers or more. But for trans- and intercontinental flights, we’ll still need to burn high-energy-density fuel of some kind.
Hydrogen
With no carbon to cast off, hydrogen offers a cleaner burn than either biofuels or synthetic hydrocarbons. It’s also easier and cheaper to make than carbon-based fuels. Paired with carbon-free electricity from sources like nuclear or renewables, it could cut the warming effect of aviation by roughly half.
The other half of aviation-induced warming comes mostly from the formation of contrails initiated by water vapor, soot and nitrogen oxides. Those emissions can absorb and reflect heat back toward Earth, contributing to climate change. Hydrogen, which produces only water when burned, may hold an advantage over other fuels in this area as well.
The net climate impact of less soot and more water vapor, however, is still unknown, and Airbus is running a study to find out. More water vapor could increase contrail formation, but the absence of soot could make it harder for clouds to form. Aerospace engineers are already exploring how to capture even the water vapor in hydrogen-powered flight, says Cesnik. Çınar says hydrogen could also be used to generate emissions-free electric power via fuel cells.
However, making hydrogen practical for widespread use comes with a daunting set of challenges. Bezos-O’Connor gave the laundry list at the Sustainable Aviation Symposium: Compressing and storing it—possibly with cryogenic systems. Insulating the system. The plumbing lines and seals. The way materials can get brittle as tiny hydrogen atoms burrow into them. Fire safety and crashworthiness. And Haverkamp pointed out that it’s increasingly recognized as a significant greenhouse gas because it keeps methane in the atmosphere longer, so leakage is more than just inconvenient.
Hydrogen becomes a liquid at about negative 423 degrees Fahrenheit, so storing it in space-efficient liquid form requires extremely cold refrigerators. This engineering problem is solved on land, with cryogenic tanker trucks that ferry liquefied hydrogen from place to place.
The stakes are higher on an airplane, though. Where does the hydrogen go if cooling fails? What kind of system is needed to protect the passengers on the plane—and get the plane to safety if its fuel supply vanishes?
Nearly every part of the hydrogen supply chain is a hurdle: efficiently producing it, transporting it, storing it at the airport and on the plane, and using it on the aircraft. However, because hydrogen is so different from jet fuel, Amanda Simpson, former vice president for research and technology at Airbus Americas, noted that it comes with interesting opportunities.
For instance, she asked, what if airports became energy hubs, producing hydrogen on site not just for planes but for applications in the local community as well? Likewise, cryogenic systems create the possibility of superconducting circuits that would offset their energy costs by providing a lossless way to move electricity around the plane, she added.
Airbus is talking about a fully hydrogen-powered passenger plane by 2035—and they may very well build one by then. But as for actually seeing hydrogen planes in the sky like we have electric vehicles on the road today, Cesnik says we’re looking further ahead, closer to 2050.
For all the challenges, Bezos-O’Connor remains optimistic that engineers can pull off hydrogen power if they decide to.
“We did this for the shuttle,” she pointed out. “We have a lot of solutions on the space side of the house.”
Making the shift
While changing consumer sentiment about climate responsibility can shift airlines’ priorities to a degree, large changes in infrastructure that require the coordination of multiple airlines and airport authorities will need the support of policy.
“It is a huge challenge,” Haverkamp said. “Government policy makers who are trying to speed up the transition need to create incentives, not try to pick winners. At this point, it’s unclear which are likely to be the most successful technologies.”
For starters, the codes for certifying aircraft need updating. The methods used to measure the available propulsion against the size of the plane, for example, don’t necessarily work for the technologies in development today.
“Have you heard about certifying one and a half engines on an airplane?” asked Susan Ying, the senior vice president of global operations at hybrid electric airplane startup Ampaire. The audience at the Sustainable Aviation Symposium responded with wry laughter.
If we consider this an urgent humanitarian issue that threatens our way of life, the cost becomes secondary.
Carlos Cesnik, Richard A. Auhll Department Chair of Aerospace Engineering
Then there’s infrastructure. Airport authorities will need new tanks and fuel lines for SAF, charge points and upgraded electrical service for electrified planes, and possibly a strategy for supplying hydrogen in the more distant future. And electricity and hydrogen infrastructure isn’t useful unless it exists at both the takeoff and destination airports.
Haverkamp also pointed out that success depends on many metrics: What is technologically feasible, economically viable, environmentally friendly and wins the support of the flying public. Above all, she says, aviation demands an extremely high safety standard. Both electric and hydrogen-powered flight pose new fire hazards. Still, it’s not as if being up in the air with more than 5,000 gallons of kerosene is an inherently safe proposition—it’s just that engineers have spent more than 70 years making it as safe as possible.
Once the technologies are available at airports, Sick thinks we can rely on wealthy fliers to kickstart the market, much as well-heeled early adopters helped EVs reach critical mass. Many of the climate-conscious rich can afford to pay three to five times the price of conventional jet fuel to fly on SAF.
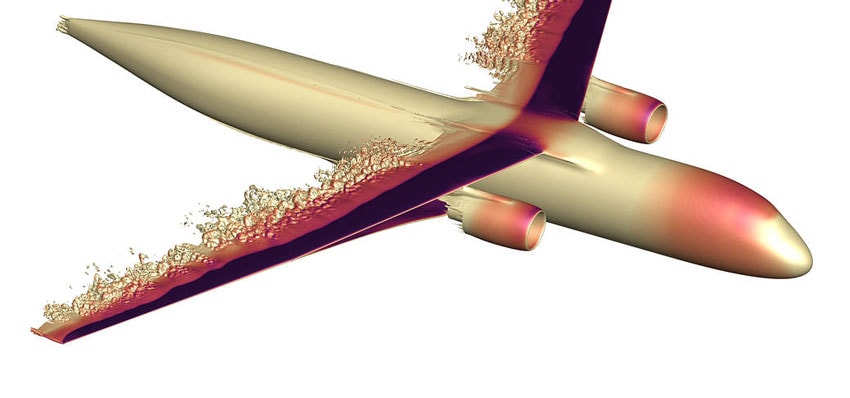
As production ramps up, SAF is expected to fall to double the price of conventional jet fuel. That extra cost could be partially offset by hybrid planes that could use 30% less fuel, and more aerodynamic designs could trim off another 10 to 20%. Ultimately, the flying masses may not see much extra cost.
Likewise on the electric front, the electric air taxis that will quickly ferry passengers to city centers are marketing themselves to the wealthy. And those who can afford their own planes, or charter services with climate-conscious clientele, are the likely buyers of the first hybrid planes.
Electrification is already getting a foot in the door at airports with air taxis beginning deployment. Entrepreneurs in electric aviation are expecting it to scale over the next decade. Still, very few engineers expect that electrification is the silver bullet.
“It’s important to understand that all these technologies have their use cases, advantages and disadvantages,” said Çınar.
The current consensus is that even if we can electrify regional and mid-range flights, we’ll still need fuels like SAF or potentially hydrogen to take us long distances. All of it is possible with investment—the challenge now is investing wisely.
“It’s a hard sell for everyone. But if we consider this an urgent humanitarian issue that threatens our way of life, the cost becomes secondary,” said Cesnik.